The modern world would cease to function without the vast, nearly infinite web of crisscrossing electromagnetic waves that travel invisibly through the air, transmitting digital data and sensing and returning information about our environment at the speed of light. In many emerging applications, such as autonomous vehicles, Lidar, and holographic displays, directing and steering light beams over time is crucial to mapping our surroundings or immersing ourselves in an augmented reality.
That is the area of photonics that interests Jon A. Schuller, assistant professor in the Department of Electrical and Computer Engineering. He wants to use specialized semiconductor materials and devices to manipulate, steer, and direct light without any mechanically moving parts, an achievement that could have wide-ranging applications.
For instance, the most-expensive component of an autonomous vehicle is the rooftop dome that houses the photo detectors and lasers that sense and direct light in time, enabling the vehicle to map and navigate its environment. Schuller is pursuing ways to use sub-wavelength interactions — defined here as involving any element smaller than the wavelength of light (about one micron) — to steer light without any moving parts. This would enable faster and more efficient sensing, making the system smaller and less expensive with better performance.
Schuller and his UCSB collaborators — Professor Chris J. Palmstrøm and PhD students Mihir Pendharkar and Prasad P. Iyer — are working with the semiconductor indium antimonide (InSb), which forms an ideal material for controlling the phase of light.
One way to encode digital data — that is, transforming letters, numbers, video, or audio into binary language that can be transmitted — is to turn a laser on and off rapidly. That can be done by splitting the laser into two waves, shifting the phase of one of the waves 180 degrees, and recombining them. As the parallel beams travel through space, the waves align but as mirror images of each other. When the peaks of one wave line up with the troughs of the other, they cancel out the signal and the laser is off. By switching the phase between 0 degrees (on) and 180 degrees (off) millions of times per second, information is encoded into the ones and zeroes of a digital data sequence.
To cause such phase shifts at the micron scale of light is much more difficult than doing so at the millimeter or centimeter scale, because, Schuller says, “At that scale, the phase-shifting device is so small that the light travels very little distance and therefore has very little time to interact with the material, so we need to find a much larger and more extreme light-matter interaction that can occur over such a small distance and time.”
The properties of indium antimonide make it useful in this regime, and Palmstrøm and Pendharkar have grown InSb crystals of extremely high quality.
In a recent paper in Nature Communications, Schuller, Palmstrøm, Iyer, and Pendharkar describe a technique for applying heat to InSb to achieve the necessary tunability of light that enters the material. “Our focus is on steering the beam,” Schuller says. “If I have multiple objects that are emitting light, then the different phases will cause the light rays to add up constructively in certain directions and destructively in others.” The light will be on in the directions identified with constructive addition and off in those affected by destructive addition.
“Our goal is to define a group of sub-wave-length elements that are essentially active mirrors, reflecting light with a controllable phase,” Schuller adds. “The goal is to be able to generate and modulate the light when it interacts with matter.”
Shifting phase at macroscopic distances is relatively straightforward. “We can use a light-matter interaction of any strength; if the interaction is weak, we just make the device longer so that the effect has more time to occur,” Schuller notes. “But once the distance is so constrained, a whole group of optical effects clearly will not work, because they are too small and therefore won’t occur fast enough. We need to look for effects that are much larger than what we can achieve with the phase shifters and modulators typically used for these processes. That's how we end up discovering some new materials science or physics.”
As an example, Schuller adds, “Let’s say we're changing a material’s refractive index by between ten to the minus three or ten to the minus four [one-tenth of a percent to one-hundredth of a percent]. That’s a fairly large change, but in this paper, we’re investigating effects that are one to ten thousand times bigger.”
The refractive index tells about a lot about how light behaves in a material, such as the angle at which light rays bend as they enter or exit, the speed of light in the material, and light’s effective wavelength in the material.
One type of modulation involves changing the number of mobile electrons in the material to change its optical properties, which in turn changes the phases. With indium antimonide, it is possible to achieve that change in electron density simply by changing its temperature, and without having to run a current through it.
Schuller explains that the thermal-free-carrier effect discussed in this paper — which refers to using heat to tune the number of electrons — the carriers is a new approach for achieving high-magnitude tuning. Free-carrier tuning is usually activated by electrical control and is not very large in most material regimes, but in InSb, the effect was found to be quite large — on the order of ten times larger than existing thermo-optic effects — and was even larger when generated by temperature change.
Ultimately, the team is trying to "broadcast," or direct, optical (infrared-frequency) waves. But unlike, say, a radio station antenna, which sends waves in all directions, they want to direct the waves in a particular direction with a high degree of control at an extremely high frequency. (Think of that sensor on the driverless car monitoring its total environment millions of times per second.) Traditionally, antennas have operated in the domain of gigahertz or megahertz. Schuller’s group is working at the scale of tens of terahertz, or 1 trillion cycles per second. “instead of doing it in the radio frequency domain, we are doing it in the optical frequency range,” he says.
For now, they are using the thermal free-carrier method to solve the challenges of optical tuning within indium antimonide. Eventually, they aim to activate the InSb electrically, which is faster and offers more benefits but is also another complex challenge. That makes the thermal approach all the more valuable, because it allows them to experiment with the material extensively without having to create the electrical system to activate it.
“This temperature technique we came up with is a clever way we can look at the basic physics of this problem, the basic optical materials science questions,” Schuller says. “We get all the complexities of the ultimate application that will use electricity.”
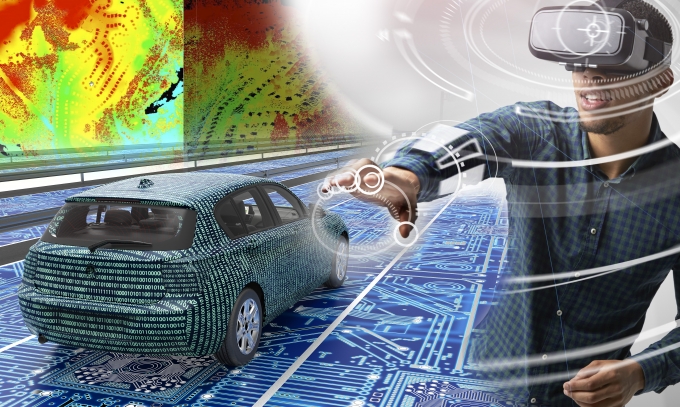
Sensing the environment more quickly and efficiently would be a benefit in such areas as remote sensing, autonomous vehicles, and holographic displays.